Disruptive Science & The Electron Microscopy Revolution
I recently met a Nobel Laureate at uni. It was an event organised by a group of structural biology students trying to get experience for their Master’s project, so the invitee was naturally an expert in their field – the cryoelectron microscopist Richard Henderson.
Albeit a rather impromptu decision, I chose to miss part of a lecture that day to atttend the guest-seminar. I had a certain question that I was really hoping to get an answer from, you see, especially if I could ask someone as involved in research as Dr. Henderson. You don’t often get to see veritable science celebrities in person, either, so I considered it a worthy trade. Suffice to say, it paid off in a most interesting and encouraging manner.
At the start of his talk, I half-expected it to be a historic account of what I had already learnt in previous lectures last term. To some extent, it was. We learnt of the conception of cryo-electron microscopy (cryo-EM) in 1981 by Jacques Dubochet, a biophysics researcher at the University of Lausanne and a rather famous contemporary of Dr. Henderson’s (hint: both won the Nobel Prize in Chemistry in 2017). We also gained insight into how scientific collaborations were formed at the time. Apparently, Henderson’s lab had been quite interested in the research being undertaken at Lausanne, so they sent one of their PhDs to train there and snatch the technique away for their own use! The issue: ‘He found it so promising, in fact, that he ended up transferring there permanently.’ I find that science can be quite comedic at times.
There were a bunch of famous EM and cryo-EM examples in the seminar, ranging from the first images taken of a virus – the tobacco mosaic virus, to be specific – to high-resolution projections of mitochondrial complex 1 and the spike protein in SARS-CoV-2. All incredibly fascinating to my fellow biologists and biochemists in the lecture hall, I imagine. What really intruiged me, however, were the last sections of the presentation. Here, I found out about the future of structural biology; about the macroscopic trends in the use of cryo-EM and X-ray crystallography in research.
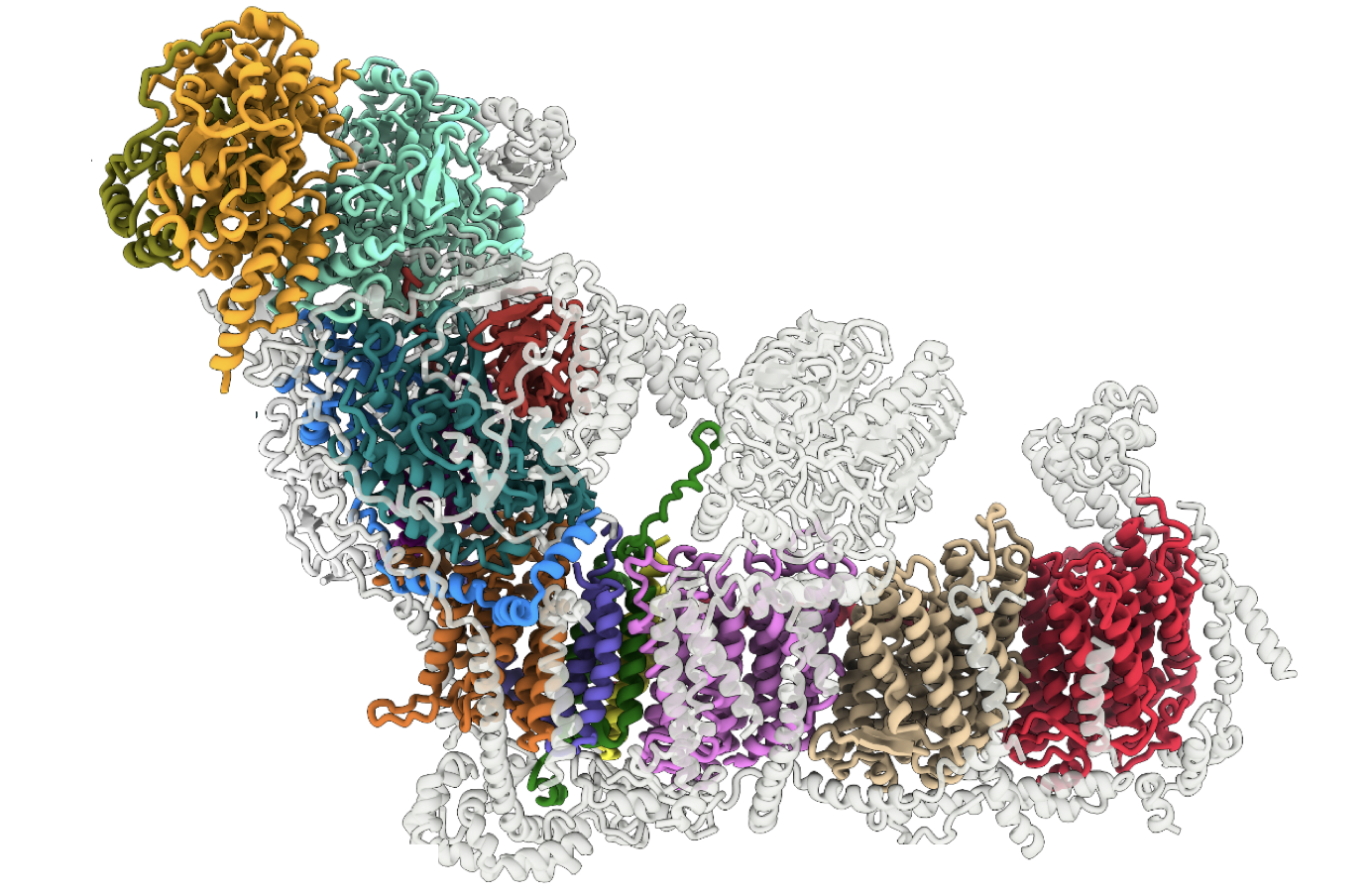
While it was not overly surprising to hear that cryo-EM is taking over, that its technological advancement beyond is plateauing was. The field is now reaching a turning point. Undoubtedly, future endeavours will find ways to circumvent technological stopgates…but for how long? And how often or significant will they be? Such ideas brought me back to my original question: ‘Is disruptive science slowing down?’
The point of disruptive science
Almost two months ago, now, Nature published an article entitled ‘Papers and patents are becoming less disruptive over time,’ discussing a newly observed decline in the degree that novel research affects technology and scientific practices today. To conduct the investigation, the researchers involved used metric indeces measuring how past inventions destabilised industrial manufacturing streams. They applied the system to 45 million papers and 3.9 million patents over the years, so the pattern they found is at least convicing. At most, it’s a real dilemma we are facing – especially if it comes from industry’s implicit demand of researchers to focus on keeping the status quo. But I digress.
There are, of course, exceptions to the study’s findings. In the last decade, the life sciences alone have made leaps and bounds in progress, with methods in the clinic already tackling what were previously thought to be incurable diseases. Every other week, a story comes out on the news about a child or elderly that was fully cured from terminal cancer with CAR-T cell therapy, made possible by recently adopted CRISPR-Cas9 gene editing technologies. Genome sequencing is also more affordable and efficient than ever, allowing researchers to scan a person’s entire genome in hours. In the case of cryo-EM, advancements have mostly focused on fixing subtle inaccuracies and inconsistent set-up procedures. We’ll see this in more detail later.
Naturally, there is currently much debate about whether disruptive science is truly ‘slowing down.’ Some argue that, even if there is truth in the idea, we may just be experiencing a threshold before research bursts with newfound potential. Indeed, with equipment like robotics, quantum computers and accelerated discovery steadily becoming more accessible to research institutions over time, we might just find ourselves overflowing with breakthrough discoveries in the near future. On the other hand, some suggest that the situation is a result of the living cost crisis affecting young researchers. Can we really expect postdocs and PhD students – the main workforce in the academia – to toil on risky, dubious, and only possibly groundbreaking research proposals when they can barely afford their own rent?
Few can say with confidence what the future will bring. Nevertheless, Dr. Henderson was quite optimistic in his talk. Even when speaking of the difficulties in convincing companies to lower their electron miscroscope prices (I wonder how he pitches that idea), he seemed certain that research would only expand in time. I am inclined to agree – especially if recent developments in imaging technology are anything to go off.
Cryo-EM taking over
Compared to other atomic-scale imaging techniques, such as X-ray crystallography or nuclear magnetic resonance (NMR) spectroscopy, cryo-EM has the distinct advantage of needing very little sample to work. When visualising a protein, for example, a few microliters of a 0.1 micromolar concentration will usually do the trick. The method also allows the sample to maintain its ‘native’ state, kept in conditions that mimic the inside of a cell before being frozen to all hell, and it is generally much faster than its alternatives.
During sample preparation, past research required you to pipette your sample onto a microscale carbon grid before plunging it into liquid ethane at -188°C. I have never used an electron microscope myself, to be sure, but I am told the prep is usually the most challenging part of the process. It can be quite finicky, supposedly. To circumvent the issue, it is now possible to use a ‘pin printer’ to add precise sub-nanoliter volumes of the sample onto the grid – just enough for recent vitrification methods. Instead of violently plunging it into liquid ethane, for instance, the cryogen may be sprayed onto the grid as minute jet streams.
Later, you must also fire the microscope’s electron beam at the sample in a vacuum, just to really make sure that it’s as dead as a boiled tardigrade (note: not actually its purpose; just an unfortunate consequence) and to image it. This involves a series of magnetic lenses that alternately focus and amplify the signal, with overly energetic electrons blocked out by apertures to increase resolution. After being deflected from the sample, the electrons are focused by an objective lens and amplified by projector lenses, ultimately hitting a detector that converts the scattered electron beam into a 2D image.
Several advances have improved the microscope itself. From the source of the electrons to how uniform the magnetic field is throughout the vacuum, the last few years have turned current electron microscopes into real spectacles for scientific research. Perhaps the most crucial step, however, has been the evolution of their detection system.
Previous experiments relied on the indirect detection of electrons, converted to rays of light to be sent through fibre optics before being interpreted by a CCD camera. Already, this was a massive improvement from the monotonous work in the pre-2000s of developing film after coating it with silver nitrate and whatnot. As much as photography prevails remarkably in the sciences, it can be a tad frustrating when you must wait hours before you can find out whether an experiment even worked. Recent direct electron detection systems are hence highly valued by the community.
In the coming years, further developments are expected to both improve and lower the costs of electron microscopes. Some promising headway from the Müller Lab at the University of California, Berkeley, is investigating the use of continuous-wave lasers as phase plates, tuning the electrons’ phase shifts for higher image contrast without unwanted scattering or charging. Dr. Henderson’s group at Cambridge is also looking into the possibility of decreasing standard protocol voltages to better resolve their images (which industry is again not particularly pleased with, as it would mean lowering the cost of their microscopes).
Clearly, there is still much potential to revamp the field. But what does this actually mean for disruptive science?
Imaging scientific breakthroughs
Rather appropriately for this post, a recent study from the University of Lausanne used cryo-EM to better understand CRISPR systems in the cell. Specifically, the authors studied how a protein called Cas7-11 binds to RNA and other ancillary molecules to form the CRISPR-guided caspase (Craspase) complex. Although their equipment was fairly traditional – using plunge rather than jet vitrification, for example – they were still able to combine their imaged projections with structure prediction programs (i.e., AlphaFold2) to build a painstakingly detailed 3D model of the complex. Later on, this could contribute into the development of useful RNA-sensing tools for the biomedical sector.
Yet cryo-EM is only one of an extensive list of imaging techniques. Even within the same discipline, we have specialised techniques like electron backscatter diffraction (EBSD), a function of scanning electron microscopes (SEMs) that provides information in microstructural analyses; or scanning tunneling microscopy (STM), which measures the distance-dependent quantum tunneling between a metal tip and a sample surface, in turn generating a signal for it. And there is an assortment of other, similarly fascinating procedures in the imaging business, both within and outside of EM.
The details of individual technologies are not too important here. Instead, it is more important to realise that researchers have a lot of tools at their disposal, many of which are even now being developed. Crucially, different tools are often also there to remedy each other’s weaknesses. Cell biology experiments don’t always need subatomic imaging, focusing more on the macroscopic observations concerning a cell’s state. This may prompt one of those underpaid postdocs to use fluorescent microscopy to visualise how a certain protein behaves inside a living cell, or to take advantage of recent non-cytotoxic super-resolution microscopy breakthroughs to identify protein-ligand interactions.
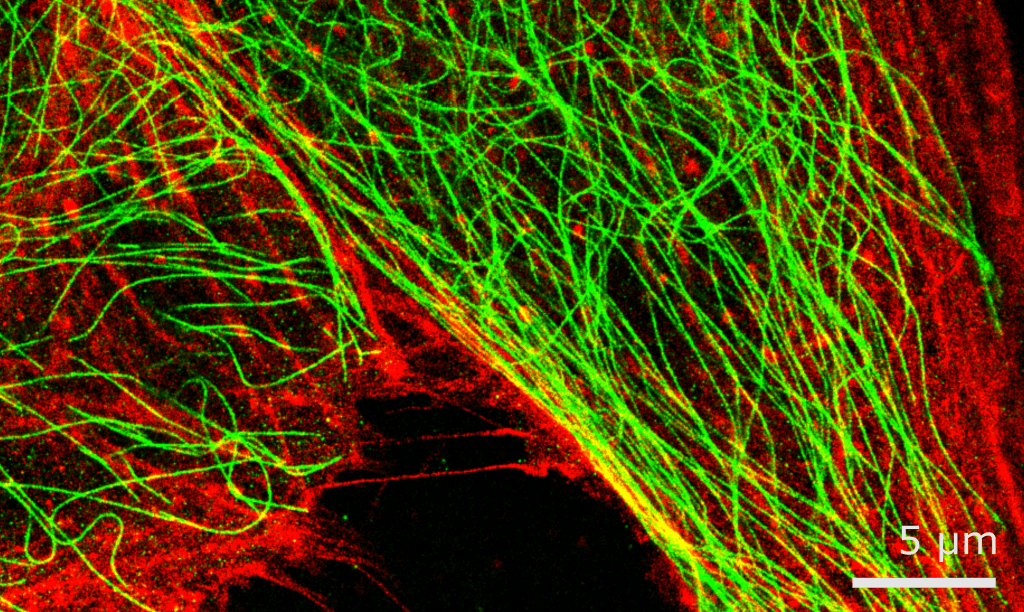
All of these techniques culminate in hundreds of discoveries every week, and no amount of metric indices or vested interests can change that. Individual studies that rock the field are probably less frequent now than they were ten years ago, even if groups like Dr. Henderson's are still making strides themselves. Nevertheless, it is safe to say that there has never been more scientific research in the world as today – and that trend won't be slowing down any time soon.
References
- Park, M., Leahey, E. & Funk, R. J. (2023). Papers and patents are becoming less disruptive over time. Nature 613:138–144. Retrieved from https://doi.org/10.1038/s41586-022-05543-x
- Assaiya, A., Burada, A. P., Dhingra, S. & Kumar, J. (2021). An overview of the recent advances in cryo-electron microscopy for life sciences. Emerg Top Life Sci 14;5(1):151–168. Retrieved from https://doi.org/10.1042/ETLS20200295
- Schwartz, O., Axelrod, J. J., Campbell, S. L. et al (2019). Laser phase plate for transmission electron microscopy. Nat Methods 16:1016–1020. https://doi.org/10.1038/s41592-019-0552-2
- Ekundayo, B., Torre, D., Beckert, B. et al (2023). Structural insights into the regulation of Cas7-11 by TPR-CHAT. Nat Struct Mol Biol 30:135–139. https://doi.org/10.1038/s41594-022-00894-5
- Fu, P., Cao, W., Chen, T. et al (2023). Super-resolution imaging of non-fluorescent molecules by photothermal relaxation localization microscopy. Nat. Photon. Retrieved from https://doi.org/10.1038/s41566-022-01143-3